France being a very secular country (well, at least it is supposed to be one), those living here end up with a bunch of catholic holidays. Life without contradictions is actually not funny, is it? As a consequence, I spent some offline time with the family last week, enjoying a four-day week-end in which I practiced some of my favourite hobbies beyond physics: having fun with my kids, gardening, outdoor time, cooking (barbecue, meat-smoking), etc.
This week is in addition quite intense. I participate to the Planck 2022 conference which is organised 10 minutes away from my office. An in-person conference is really nice as it offers the possibility to meet again friends and colleagues I haven’t seen for a long time… Let’s hope an in-person Hivefest will eventually happen later this year. As promised to @gentleshaid, I’ll try to grab some muons from the conference and share them with everybody on Hive.
As a consequence to all of this, my activities on chain slowed down a bit and turned out to be delayed (I apologise to anyone still waiting for a reply). In addition, my weekly blog on particle physics is over-due, as well as an update relative to our citizen science project on Hive. Moreover, I still owe a few French versions of my blogs to the Hive French-speaking community. I promise: later this week (or next week), everything should (will?) get back to normal.
In the meantime, Hive brought me to Twitter, and some of my physicist contacts there brought to my attention an article addressing dark matter models suitable for investigations at particle colliders (like CERN’s Large Hadron Collider). After thinking a bit, I decided to dedicate a blog (I mean: this blog) about how simplified models are actually built. The reason is that this actually brings complementary pieces of information to the blog of last week, which addresses one of the darkest secrets of the universe (i.e. dark matter) and how to get insights on it at particle colliders.
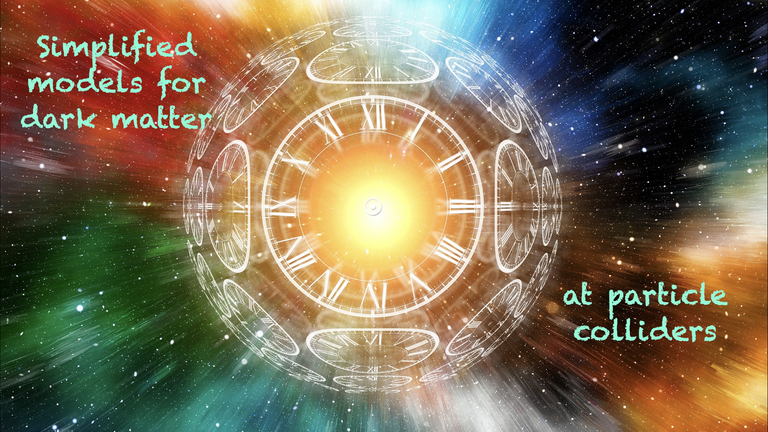
[Credits: Original image from geralt (Pixabay)]
In the next part of this blog, I first provide a small recap about dark matter. For longer (non-recap) versions of this recap, please see this good old blog, as well as the post of last week. After this, I discuss the main details of the topic considered this week, and introduce an example in which a simplified model suitable for dark matter searches at particle colliders is built.
As usual, those in a hurry could move on directly with the summary section of this blog, and the others could just buckle up and chill.
Dark matter, dark matter, dark matter… Why?
Usually, when it is time to introduce dark matter, I jump straight to key motivations. However, by doing so I forget mentioning very important fine-prints (as correctly pointed out by @stayoutoftherz in the discussion of the previous blog). Therefore, let’s start with this today, for a change!
Since 100 years ago, many cosmological observations challenge our understanding of the manner our universe works. Finding an explanation for those gave rise to the so-called standard model of cosmology. Such a model has the particularity to be extremely simple (simplicity is always great when it works), and to feature very few free parameters, dark matter and dark energy.
We can fit the model to data and see that it explains very well a lot, although some anomalies are (reasonably) in the way. Whereas the standard model of cosmology works well, it is important to stress that this is a model and that it lacks a physical foundation. Other options exist and are not excluded. However, they do not provide a fit to data that is as good (which is why the standard model of cosmology is so appealing and acknowledged by most).
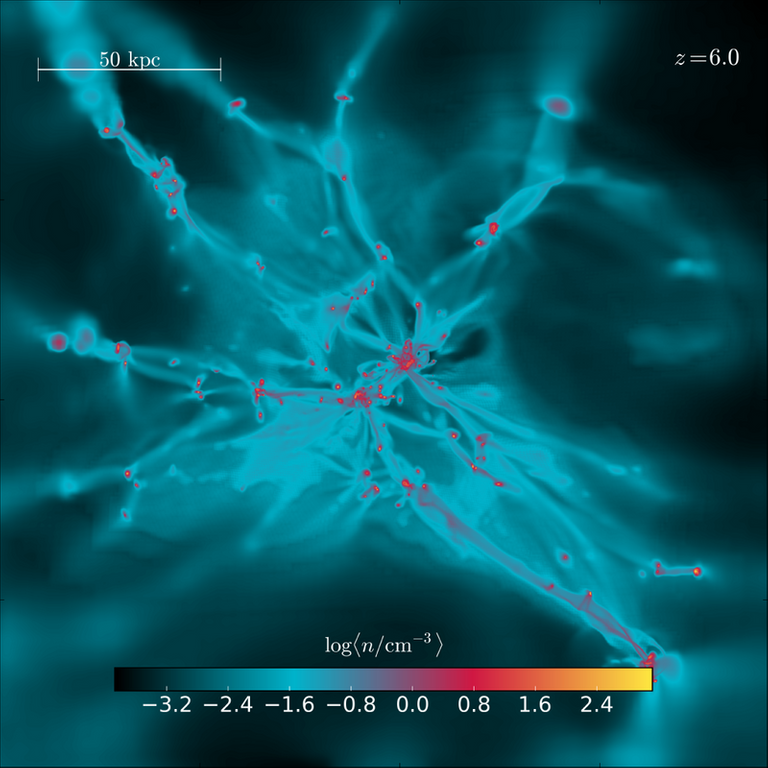
[Credits: Vis-sns (CC BY-SA 4.0)]
To summarise, the standard model of cosmology consists of one model, few parameters and many explanations to observed phenomena.
Those observations include for instance galaxy rotation curves. The latter show that stars lying very far from galactic centres rotate much faster than what is expected from gravity and the amount of visible matter in the universe. Something may be there, invisible and waiting…
Whereas this was one of the initial facts that has led to our understanding of cosmology as it is today, we cannot ignore the cosmic microwave background. It consists of a fossil radiation left over from the time atoms formed, and it provides a very precise footprint of the universe at this time (i.e. 380,000 years after the Big Bang). The standard model of cosmology fits the observation very neatly!
Moreover, standard cosmology describes quite well the structure of the universe in terms of how galaxies are organised in the universe. This is illustrated on the image above, although this only shows the universe when it was a fresh little boy of 1,000,000,000 years old.
Colliders and dark matter - a supersymmetry-inspired model
Whereas the standard model of cosmology and its successes motivate the assumption of dark matter, we have no clue about the nature of the latter. For that reason, anything (okay, mostly anything) is allowed. This does not render the situation practical from a particle collider standpoint, as we end up with gazillions of model that should be tested. In other words, particle physicists are… outnumbered!
For that reason, we designed a few simplified models that are representative of many complete and well motivated setups. This is precisely one of the ideas which I have tried to convey in my last blog. Moreover, among all existing simplified models (we have a small number of them on the market), some of them have strong underlying motivations.
For instance, supersymmetry consists of one of the most popular class of theories beyond the Standard Model of particle physics. I won’t spend time in detailing what is supersymmetry, why it is great and why it is a nice way to solve some of the conceptual limitations of the Standard Model. If this is of any interest to you, please follow this link. This is not needed for the following.
I instead simply consider one of the most common setups arising in supersymmetry and that is capable to provide a particle responsible for dark matter in the universe. This last sentence contains a strong assumption. Whereas we so far accepted the idea of dark matter, we now must admit that there is an elementary particle related to it. In addition, in the supersymmetric context (as well as in many dark matter models), the dark matter particle is connected to the Standard Model.
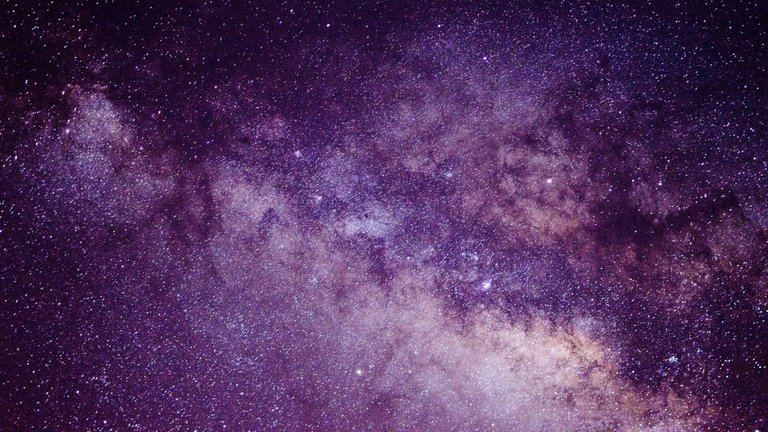
[Credits: Jason Jacobs (CC BY 2.0)]
This connection is not a direct one (this originates from the fact that a dark matter particle has to be stable), but goes through an intermediate particle playing the role of a heavier mediator. Supersymmetry provides us various candidates for such a mediator, each option differing by how the mediator connects to the quarks or the leptons of the Standard Model.
In order to design our simplified model for dark matter, we thus begin by following a supersymmetric inspiration. This defines a dark matter setup in which we have a dark matter particle that connects through a mediator to either the quarks of the Standard Model, or to the leptons.
In terms of free parameters, we therefore have two new physics masses (that of the dark matter and that of the mediator), and the theory predicts the value of all new couplings that dictate the connection between the mediator, the dark matter and the Standard Model. The next step is to relax this and make the couplings free parameters.
So we have a great simple model, at least in the sense that it has dark matter and finds its roots in a concrete well-motivated framework. However, is this viable? This question is addressed in the publication discussed today.
The incredible bulk
Let’s first emphasise again a very important point.
Whereas inspired by supersymmetry, the above simplified model for dark matter phenomenology is more general than the initial supersymmetric model. In supersymmetry, the new couplings are predicted to be equal to some value. On the contrary, in the simplified model we can fix them to any value. This is the power of simplified models, somehow. We start from a motivated framework and then generalise it.
A typical and concrete example of a motivated realisation of the simplified model consists of the so-called bulk region of the minimal supersymmetric parameter space (cf. the subtitle of this section). Here, the dark matter is connected to the Standard Model through hypercharge interactions.
I promise, ‘hypercharge’ is an existing word. However, I don’t enter into details as this is not the subject of this blog. Instead, I only mention that hypercharge interactions can be seen as admixtures of electromagnetic and weak interactions (two out of the four known forces). That’s all.
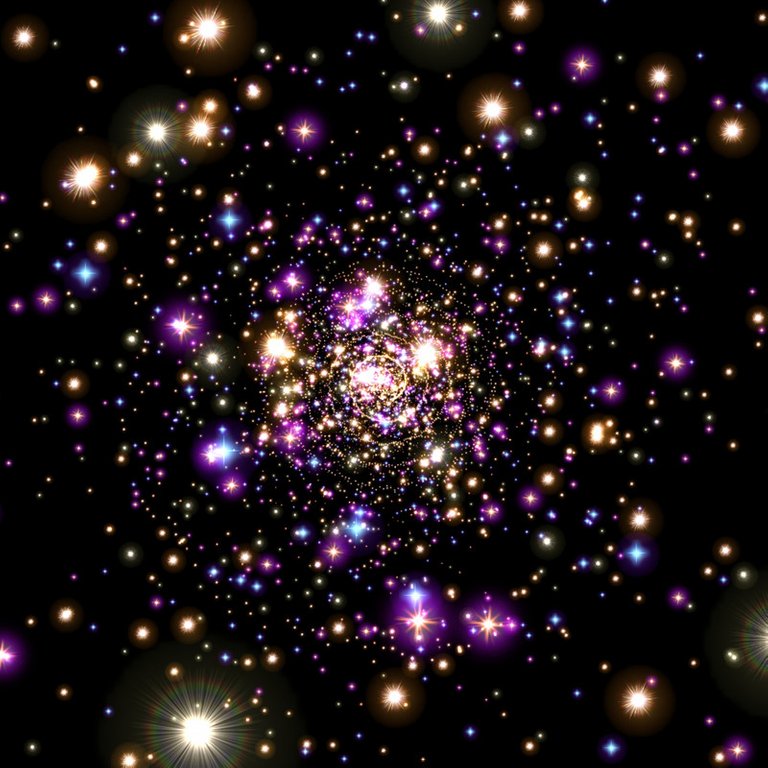
[Credits: Piotr Siedlecki (public domain)]
There is however an important consequence when we calculate the amount of dark matter present in our universe. In this bulk configuration of the simplified model, it turns out that enforcing predictions and data to agree leads to mediators that are light particles.
This is a big problem. The Large Hadron Collider is looking very widely for new phenomena and new particles, and it has not found any signal (yet?). There is now way guys and girls like our mediators could have escaped detection.
Supersymmetry-inspired dark matter at all costs!
This leads to a weird contradiction. We have a simplified setup whose most typical realisations are excluded by data. There are however three ways out (pffffeew), and it is important to consider them all and not sending anything to the graveyard.
The first one is to say that we don’t care. The simplified model is more general than the initial model and allows for exploration on general grounds (as all new masses and couplings can be tuned freely). It is thus not a big deal if we probe signals living far from any motivated model configuration. If by any chance there is a signal in data, then it will be the time to connect the simplified model to something more concrete (and we can trust physicists to design hundreds of ways…).
There are however to more physically sound options to make the simplified model an interesting avenue. These are more ‘top-down’: we start from the complete theory and try to see under which conditions the simplified model can survive. From there, we can somehow define favourite regions of the model’s parameter space, and we can study the associated phenomenology.
One of these options fits in the so-called non-minimal path that I discussed in the blog of last week. An extension of the mediator sector of the theory is sufficient to get the right amount of dark matter. Of course, we don’t do this blindly (physics must be solid!). We use instead the fact that in any supersymmetric model many new particles lie around. It is then sufficient to decide that a few of them are relevant.
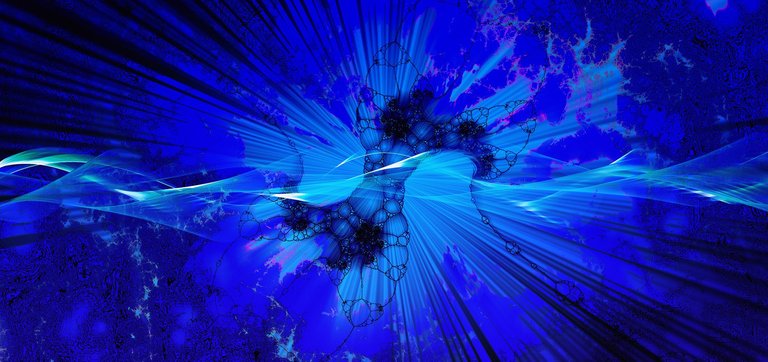
[Credits: geralt (Pixabay)]
For the last option, the idea is to keep the content of the initial simplified model minimal, and instead make the mediator only slightly heavier than the dark matter. This particle spectrum is said to be compressed. A direct consequence is that the mediator plays a much greater role in the early universe, and it thus naturally impacts the amount of dark matter left today.
Let’s put ourselves at the very beginning of the history of our universe. The amount of dark matter that is observed today stems from processes that were occurring at that moment. In particular, the key process is that in which a pair of dark matter particles annihilates into a pair of Standard Model particles, together with the reverse reaction (the production of a pair of dark matter particles from the annihilation of two Standard Model particles).
If the mediator is close in mass to the dark matter, then extra reactions must be considered. These include the production of a pair of mediators, as well as the associated production of one mediator and one dark matter. Of course, the reverse processes are important too.
Therefore, if we have a configuration in which the dark matter and the mediator have almost the same mass, we must account for all above-mentioned processes. Then, we naturally obtain an amount of dark matter in agreement with data, making the model viable.
From there, we need to investigate the consequences of those viable scenarios in the context of all present and future experiments. This allows us to see where we go, and also which probes of new phenomena are missing. Equivalently, we need to answer the question about the configurations of the model uncovered, and how to fill those gaps. This is however not the topic of this blog.
Summary: building simplified models for dark matter at colliders
In this blog, I discussed once again dark matter, in the context of the models we use to probe it at particle colliders.
The starting point of our story is the so-called standard model of cosmology that is simple and that allows for an explanation to many phenomena observed in the universe. This model has a very cool property: there is an invisible substance called dark matter. The next question to answer (in this context) is thus about the possible nature of dark matter. What is it exactly?
If we assume that there is an elementary dark particle that plays the role of dark matter and that interacts in some way with known particles, then we end up with a game to which particle colliders can contribute. This however requires to simulate signals, which is an impossible task due to too many potential models and theories featuring dark matter. For that reason, particle physicists came out with simplified models.
In this blog, I explain how such a simplified model could be constructed. The starting point is a well motivated framework for physics beyond the Standard Model, like supersymmetry. In such a model, we have a particle that can play the role of dark matter, and it comes together with a connection to the Standard Model. This connection occurs through mediator particles. The next step is to remove everything from the model, excepted the dark matter and the mediator.
Now, we can do some calculations and verify under which circumstances the amount of dark matter is the correct one relative to data. And this is where it becomes tricky: the mediator particle must generally be light, which is in contradiction with its non-observation at CERN’s Large Hadron Collider.
We are thus offered three ways to move on.
- We generalise the model by making the predicted couplings free, and use the new ad hoc setup for exploration.
- We make the model non-minimal by complicating the mediator sector on the basis of what supersymmetry allows us to do, so that we could fit the right amount of dark matter and be compliant with experimental bounds on new particles.
- We keep minimality and focus on an option in which the mediator is only slightly heavier than the dark matter. In this case, more processes are relevant in the early universe, allowing to get the right amount of dark matter together with a heavy enough mediator.
It is then up to us, physicists, to study the phenomenological implications of the three setups and make sure all options are covered in present and future searches. The major issue is not to waste any possibility to discover something new!
I stop here for today, and I hope you all enjoyed this blog. I would be happy to read your comments, feedback and suggestions. In addition, please be ready for a change of topic soon (neutrinos). Note that I may skip next week as another long week-end is in order (France, France, France).