This week is a special week at STEMsocial. It is our dark matter week, with a special event to be announced tomorrow (i.e. on Tuesday). The only thing I say for now is that this event is open to everyone, which includes in particular those not versed in science. To know more about this, please follow our community account @stemsocial, and stay tuned for a few more hours.
In the meantime, I take the opportunity to discuss some important generalities about dark matter, complementing the partial information provided in my last two blogs (here and there).
I begin the current blog with a short presentation of what dark matter is and where the idea came from (see previous blogs for more crispy details). I then describe how dark matter is searched for by high-energy physicists from all over the world, and try to share the reasons of my excitement as soon as light is shed on this dark topic (I even try to be funny… that’s probably a side effect of dealing with COVID@home).
For those pushed for time, please consider moving directly to the last section of this blog. As usual, feel free to ask questions, not only on the topic of this blog but also about particle physics in general. Who knows, maybe this will give me extra ideas for the next blogs (on top of the hundreds of topics that I already want to cover).
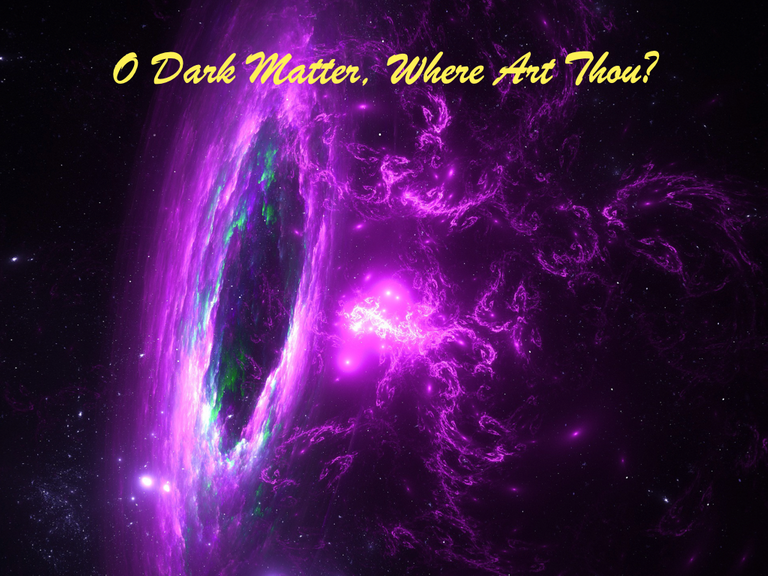
[Credits: Original image from Maklay62 (Pixabay)]
An old dark relic in our universe
As already mentioned several times in my posts, phenomena around dark matter consist of a hot topic in high-energy physics. Whereas dark matter is very motivated by almost 90 years of observations in the sky, the Standard Model of particle physics (that itself accommodates a wealth of data) is not capable to do anything for dark matter. These two points taken together are the source of our excitement when dark matter is at stake: there must definitely be something beyond the Standard Model.
However, where is dark matter coming from? Why do we believe there is such a weird substance in the universe? And what is this substance after all?
The story starts in the 1930s with observations made by the Swiss physicist Zwicky. Zwicky reported measurements of the rotation speed of stars in a distant galaxy, and confront them to predictions from the theory of gravitation. He found out that stars lying very far from the galactic centres rotated too quickly relative to expectations. To accommodate observations, it was postulated that some invisible matter hid itself in galaxies, and was responsible for this too fast motion of stars.
Together with a robust confirmation of these observations in the end of the 1960s and beginning of the 1970s by Rubin, these conclusions are the first proof pointing to the existence of some matter that was different from all known matter. This difference is this darkness, dark matter being dark in the sense that it is transparent to light (in order words, it ignores light). The proof is however indirect.
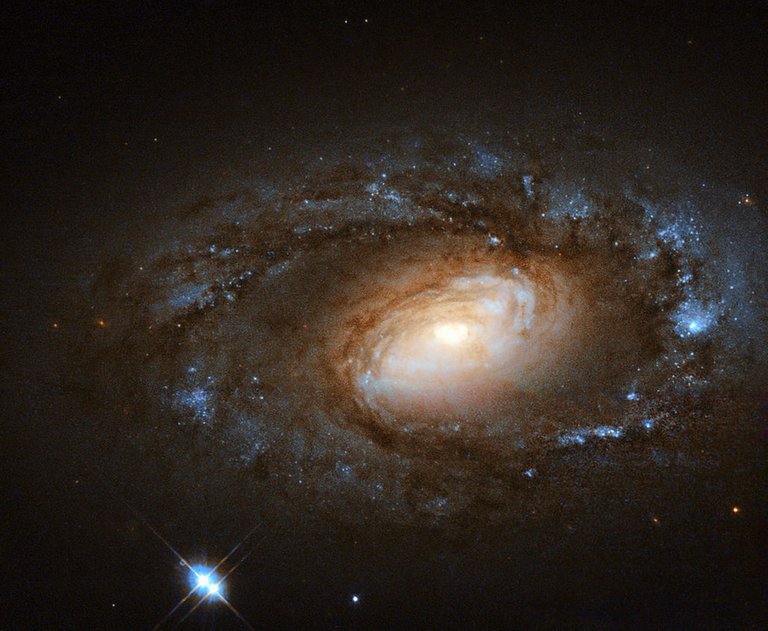
[Credits: NASA (CC BY 2.0)]
To summarise where we are so far, anomalies in the motion of stars in galaxies where found in the 1930s and confirmed in the 1970s. In order to provide an explanation without enforcing Newton’s theory of gravitation to be incorrect, we need to assume that some invisible matter is present in our universe. Is this sufficient as a justification to get excited by dark matter? Probably not, which is why there is more!
In 1965, Penzias and Wilson accidentally discovered that the universe was entirely filled with a radiation whose temperature was 2.73 degrees Kelvin (-270.4 degrees Celsius). We know today that such a radiation, called the cosmic microwave background (or the CMB for short), is well explained by standard cosmology. The important moment in the history of the universe is about 380,000 years after the Big Bang.
Before that key moment, atomic nuclei and their surrounding electrons were not bound together. Matter was therefore electrically charged, the nuclei being positively-charged and the electrons being negatively-charged. Radiation cannot therefore travel much, as it scatters on anything that carries an electric charge (and electrically-charged objects are just everywhere in the universe).
What changed 380,000 years after the Big Bang is that the universe cooled enough to allow nuclei and electrons to form neutral atoms. This changed the game, as radiation got allowed to travel without any limitation in space (there were no more charged objects in the universe). Radiation from that time (i.e. the CMB) is thus still mostly there today, and can be studied.
Precision having improved relative to 1965, we know today that the CMB has not a constant temperature of 2.73 degrees Kelvin. This last value is instead the average CMB temperature, tiny variations in the CBM temperature having been observed. These variations provide a map of what the universe was 380,000 years after the Big Bang. The conclusions obtained from this map are irrevocable: we need dark matter.
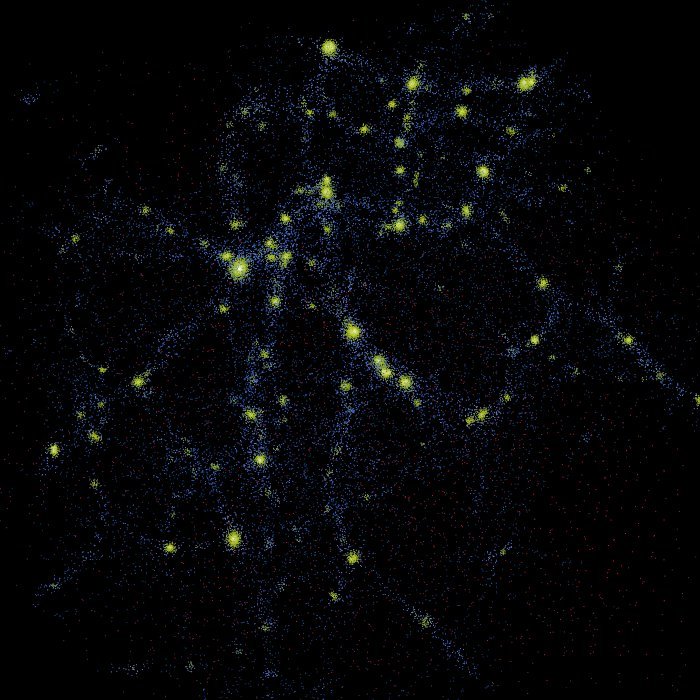
[Credits: Michael L. Umbricht (CC BY-SA 4.0)]
This need can however get wilder. Without dark matter the structure of the galaxies in terms of the stars they contain, and the structure of the universe in terms of the galaxies it contains, would indeed be different from what we observe. In other words, the structure of our universe as it is today requires the presence of dark matter.
We can also mention gravitational lensing as an other indirect proof for dark matter, as the presence of dark matter everywhere in the universe influences the way light travels (and this can be measured).
So, there must be dark matter in the universe to accommodate a bunch of observations. These are however all indirect evidence, and a direct observation of dark matter is still missing. What I want to discuss in the rest of this blog is what physicists do in order to fill this gap, catch dark matter and observe it in all its glory details.
The almighty graph of dark matter experimental searches
Existing searches for dark matter rely on two assumptions. First, we need an elementary particle that can play the role of dark matter. Second, dark matter is expected to interact with the Standard Model of particle physics, on top of its gravitational interactions.
The way to realise this in a concrete model was explained in this earlier post. For the present blog, let’s avoid any related subtleties and consider instead that we have a dark matter particle that interacts with the Standard Model.
This dark matter particle and its interactions with the Standard Model pave the way to processes involving two dark matter particles and two Standard Model particles. These processes are the roots of all on-going searches for dark matter. This connection is illustrated in the beautiful (hmmm…) homemade figure below.
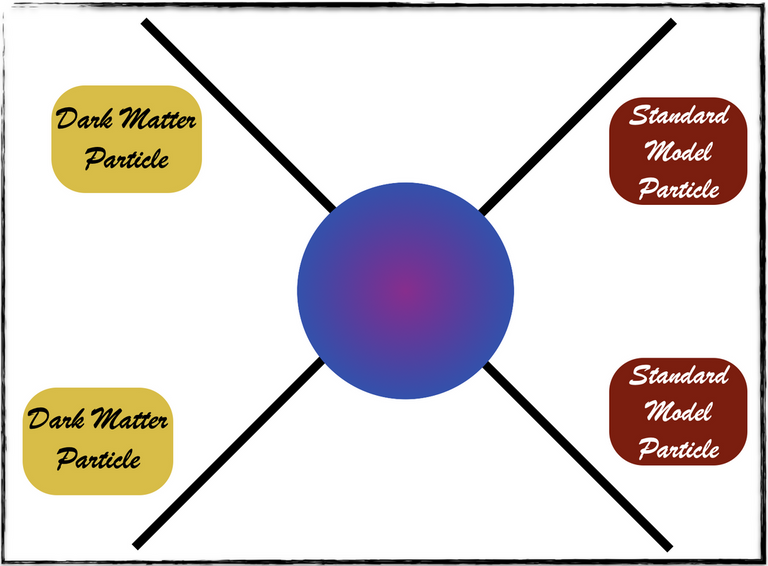
[Credits: @lemouth (this can be guessed I imagine…)]
What can we see in this figure? That’s quite simple. We have two dark matter particles (the thick lines on the left of the figure) that can react in some way (the coloured blob in the middle) and produce two Standard Model particles (the thick lines on the right of the figure).
However, this figure has more physics inside. We can have a look at it from the right to the left. In this case, we would have two Standard Model particles that would react to produce two dark matter particles. This is the inverse reaction of the previous one.
But there is even more. We can additionally have a look at this figure from the top to the bottom (or to the bottom to the top; that’s the same). In this situation, we have one dark matter particle and one Standard Model particle (the upper part of the figure) that scatter, and this gives rise to the same dark matter particle and the same Standard Model particle in the final state (the lower part of the figure), although with different kinematic properties.
This graph can thus be read in three different manners, which correspond to the three existing ways to search for dark matter experimentally.
A three-fold way to search for dark matter
It is now time to explain how the above diagram can be used to directly discover dark matter, which naturally brings us to the main meat of this blog. The first manner I discuss is when the graph is read from left to right. We thus consider a process in which two dark matter particles annihilate into two Standard Model particles.
In order for such a process to happen, we need to focus on regions of the universe that are dense in dark matter, so that we could actually have two dark matter particles that can get to know each other very closely. Such regions exist (which is a good news for the considered process to happen). They include, for example, the centre of the Milky Way or dwarf spheroidal galaxies, i.e. galaxies with very little stars and mostly made of dark matter.
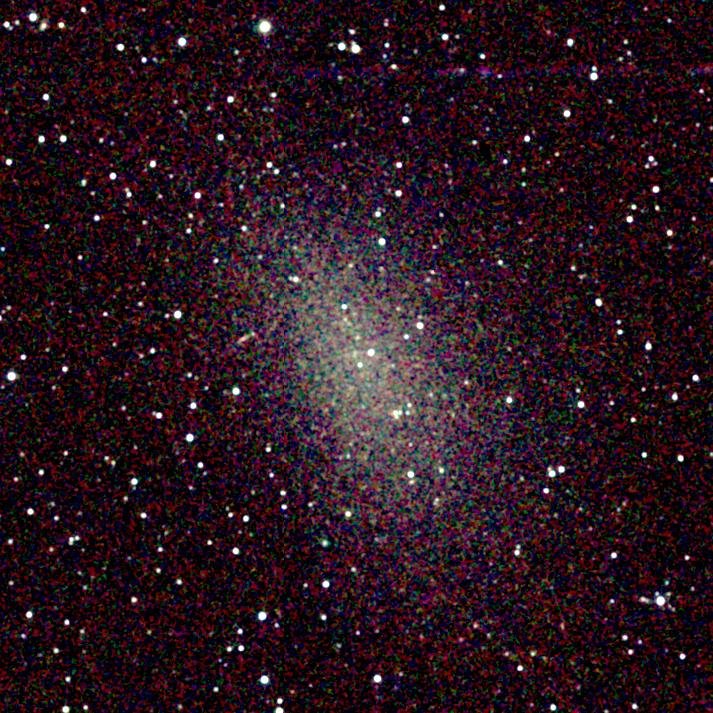
[Credits: 2MASS (public domain)]
There are therefore regions of the universe in which Standard Model particles can be produced from dark matter annihilations. Once produced, those final-state Standard Model particles contribute to the flux of cosmic and gamma rays traveling into space. These can be detected by satellites orbiting around Earth, as for instance with the Fermi-LAT telescope which measures the flux of gamma rays reaching Earth as a function of the gamma ray energy.
In order to find a signal of dark matter, we compare the observed fluxes of gamma or cosmic rays arriving on Earth with expectations from various astrophysical sources (i.e. the background to our signal). No excess relative to astrophysics has been found so far…
The second way to seek dark matter on the basis of the above diagram is to read it from right to left. As already mentioned, this is the reverse process of the one giving rise to cosmic and gamma rays. Here, two Standard Model particles annihilate and give rise to the production of a pair of dark matter particles.
As dark matter particles are often considered much heavier than any Standard Model particle, the latter must be accelerated to enable dark matter production. This stems from Einstein’s theory of special relativity. Mass is a specific form of energy, so that more energy (obtained through particle acceleration) is needed to ensure that heavier particles could be produced.
The associated experimental context is exactly the one put in place in big particle accelerators such as the Large Hadron Collider at CERN. An example of collision in which dark matter could have been produced is shown in the figure below.
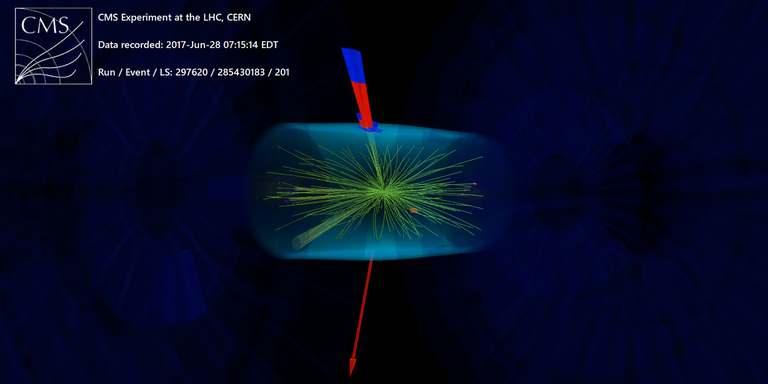
[Credits: CERN]
The interesting question is definitely about how can we relate the above detector record to the production of dark matter? This comes from one of the golden rules in physics: energy and momentum conservation. In any process, these two quantities are conserved. That’s a fact.
Consequently, this can be verified in any collision. Let’s take the above figure. We account for all energy deposits (the red and blue hits in the upper part of the figure) and all tracks (the green lines in the middle of the figure), and then check whether energy and momentum are conserved. Interestingly enough, we can observe that something is missing (the red arrow in the lower part of the figure). Such a missing energy and momentum is associated with particles leaving the detector invisibly (for instance because of their very feebly-interacting nature).
Missing energy can thus be linked to dark matter production, as dark matter is very weakly-interacting and would leave a detector invisibly if produced. This process is however not a background-free process, as a missing energy signal could come from the Standard Model too.
The results of all analyses expecting to find dark matter are for now compatible with this Standard Model background. No signal again…
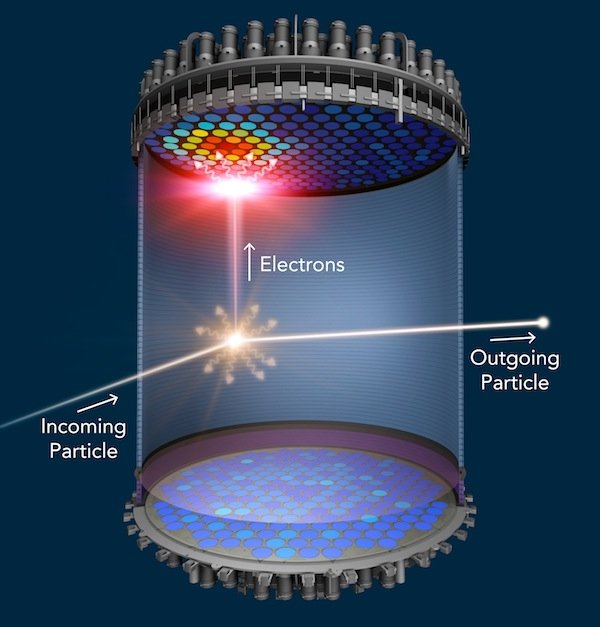
[Credits: SLAC]
It is now the time to discuss the last way to probe dark matter, when our almighty diagram is taken from its top part to its lower part. This process can be seen as a reaction in which a dark matter particle hits a Standard Model particle at rest, scatters and generates the recoil of the Standard Model target. This phenomenon is exploited in dark matter direct detection experiments (see the above picture).
Recent examples of such direct detection experiments include the Xenon-1T detector. In this case, we have a huge tank filled with liquid xenon, and we patiently wait as this tank is traversed by a wind of dark matter constantly blowing on our planet. When a dark matter particle of the wind hits a xenon atom (its nucleus or one of its orbiting electrons), a small recoil can be measured.
Whereas such an event is very rare (due to the weakly-interacting nature of dark matter), we have a huge detector (1 ton of liquid xenon, that will soon be upgraded to several tons of liquid xenon). Therefore, by waiting during a sufficiently long time, we can expect to see some events.
Unfortunately, once again… no signal has been found. Dark matter is hence much more elusive than in most optimistic scenarios.
I didn’t have time for such a long post... What is all of this about?
Dark matter is probably the most elusive substance ever. We know it has to be there thanks to various sources from cosmology: galaxy rotation curves (i.e. the motion of stars around galactic centres), the cosmic microwave background or the formation of the large-scale structures in the universe. However, these strong motivations are all indirect, and dark matter still needs to be directly produced and observed.
We are looking for this in three different fashions, all originating from the fact that if there is a dark matter particle and if this particle interacts with the Standard Model, we automatically have reactions involving two Standard Model particles and two dark matter particles.
- Two dark matter particles can annihilate into two Standard Model particles. That gives rise to cosmic ray and gamma ray signals that can be observed in satellite experiments orbiting around Earth.
- Two accelerated particles can annihilate to produce dark matter. This is the strategy followed at particle accelerators that seek a dark matter signal (i.e. a missing energy signal) in data.
- One dark matter particle can hit some Standard Model particle and make it recoiling. Observing such a recoil is what direct detection experiments aim to do.
Despite of this variety of searches, dark matter still escapes us today. Most proposed dark matter models in particle physics therefore get more and more constrained. However, there is still room for dark matter to be viable, and the future is coming with great expectation (new experiments, more data, more precision, etc.).
I hope this introduction, which I end here, was interesting enough. Please feel free to ask questions (on this blog or on particle physics and cosmology in general), and provide feedback (both positive and negative ones) in the comment section.
I wish everyone a very nice week, and see you tomorrow from @stemsocial for a special announcement related to dark matter!