At the end of my talk at HiveFest, I showed some scientific results about dark matter. I mentioned that they were related to the topic of one of my future blogs, and to that of one of my future scientific publications. This publication was released on the arXiv last week. Correspondingly, a blog is due!
Today I discuss composite models as potential solutions to some of the conceptual and practical limitations of the Standard Model of particle physics. For general information about the problems of the Standard Model, please see here and there. In the present blog, I only discuss what composite models are, and I obviously provide a brief recap about dark matter. Then I enter the main topic of the blog: my last publication about composite dark matter.
In this article, we found that composite dark matter scenarios may be easier to observe than expected. Whereas experiments targeting dark matter direct detection (I explain what they are below) were known to be mostly insensitive to such scenarios, these conclusions relied on a too simplistic description of the theory. We instead demonstrated in our article that future experiments have a chance to observe some composite dark matter models.
If you are pushed for time, please consider going directly to the summary given at the end of this blog. Otherwise, please buckle up and enjoy some particle physics.
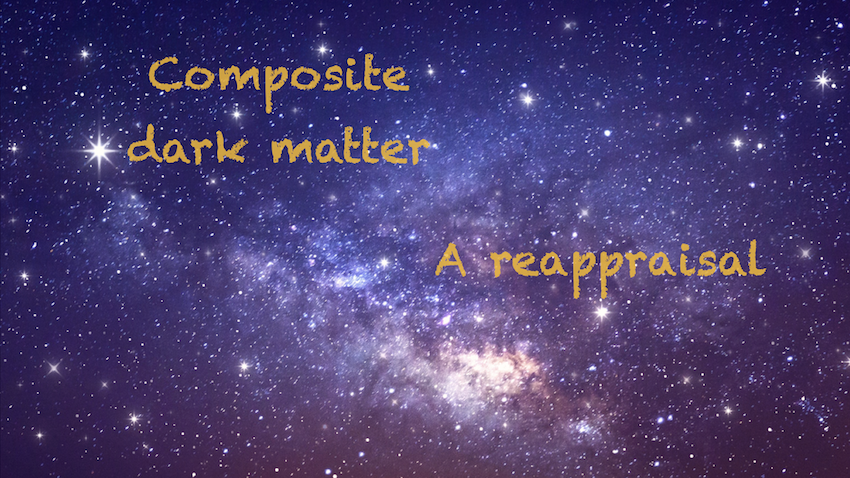
[Credits: Original image from Andrea Stöckel (public domain)]
Composite models for new phenomena?
A composite theory is a theory in which new fundamental building blocks of matter are postulated, and these building blocks are strongly interacting with each other. Consequently, they form composite particles. At energy scales currently probed, for instance at CERN’s Large Hadron Collider (the LHC), these fundamental particles are too heavy to be observed. However, there is a vast zoo of composite objects that are potentially lighter, and thus reachable at particle colliders.
This idea is not new, as it is the one that lies behind the theory of the strong interaction, one of the three fundamental forces described in the Standard Model. In this last case, quarks (the fundamental building blocks of matter) form composite particles called hadrons (like protons, neutrons or pions). Designing high-energy experiments for more than 50 years allowed us to break hadrons, and get some insights on their quark content.
A similar story holds for composite models (assuming that nature picks them as its favourite option for physics beyond the Standard Model). However, the energy needed to break the composite objects and to access their internal structure has to be much larger than any energy regime that can be reached in current experiments.
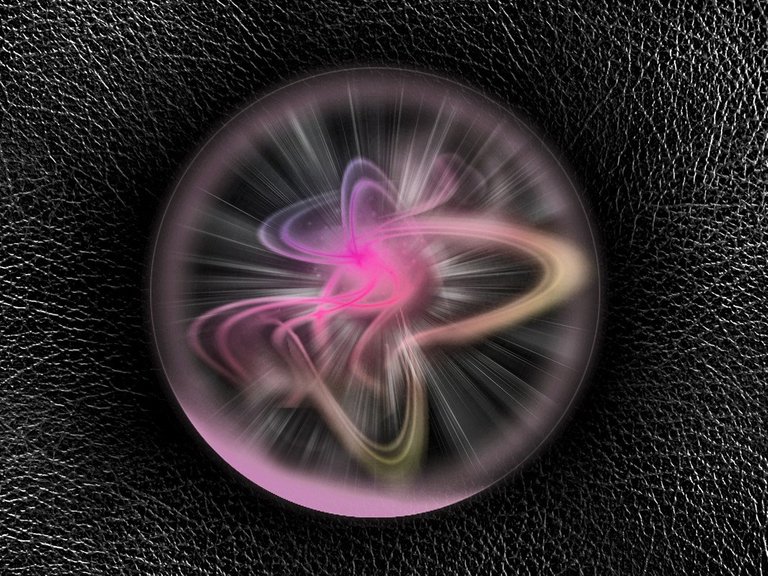
[Credits: ThomasWolter (Pixabay)]
To repeat, composite models have the particularity to predict a vast zoo of new particles that are combinations of a small set of fundamental particles. A few of those states could be very light, and thus potentially observable at the LHC. The lightest of those states is the Higgs boson, which is allowed by data to have a composite origin. Note that on the other hand, there is no proof (yet?) that the Higgs boson is composite. It is just a possibility that has not been ruled out (yet?).
In addition to the Higgs boson, other light states may be around the corner, and accessible at the LHC. One of them could be a good candidate for dark matter (assuming that dark matter is associated with a particle; see below), and another could be a mediator linking dark matter to the Standard Model. This mediator is generally related to the top quark, the heaviest of all elementary particles known.
The reason behind this new particle configuration is that composite models encompass a mechanism called partial compositeness, that is needed to explain the large mass of the top quark. This somewhat promotes the top quark, which then plays a special role in the theory.
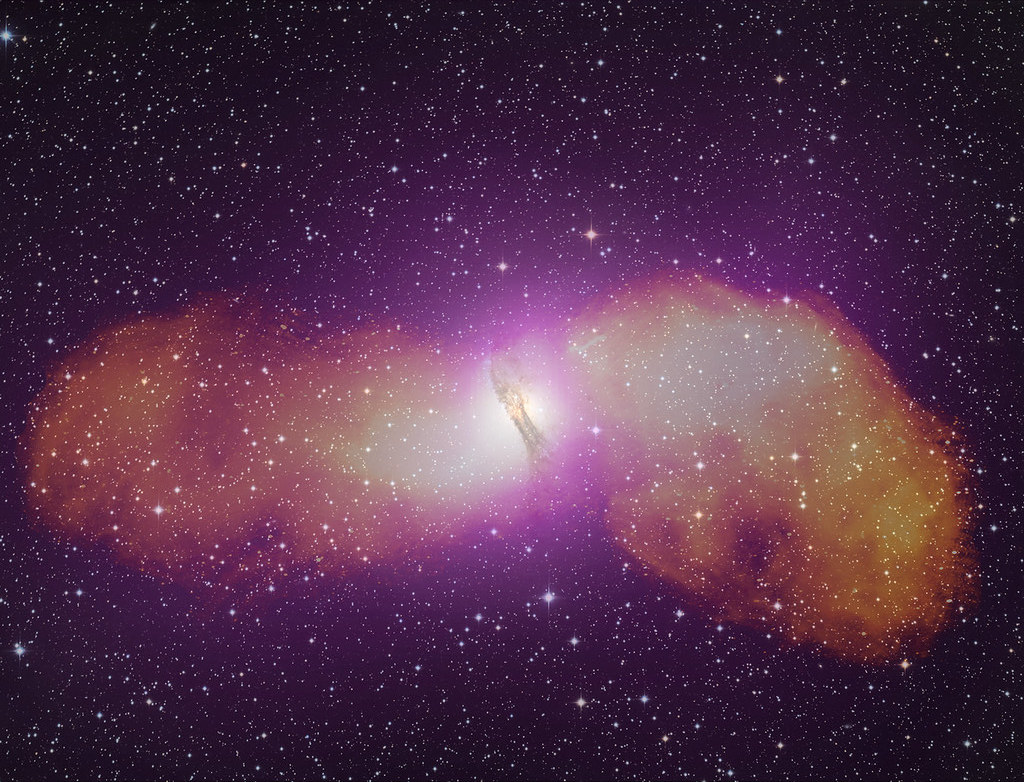
[Credits: NASA (CC BY 2.0)]
Dark matter and how to search for it
Dark matter consists of a very popular topic in particle physics and cosmology today, as it is probably one of the most elusive substances for which we ever searched.
The origins of dark matter can be found in discrepancies between theory and data in the context of the rotation motion of stars in galaxies. Fritz Zwicky (and Vera Rubin a bit later) found that stars were moving too fast compared with predictions of classical mechanics. Two options are thus left: either some mass is invisible (i.e. dark matter) or classical mechanics needs to be modified at large distances. We adopt the first option.
Secondly, we can imagine that dark matter must have a particle physics explanation. In other words, there must be a particle that can play the role of dark matter. That is our second assumption. The good news is that these two assumptions provide an explanation to several cosmological observations: the cosmic microwave background, the formation of the large structures in the universe, gravitational lensing, etc.
I won’t enter into all these details, as they were already provided in many of my earlier posts. What is important to remember is that dark matter allows us to solve many puzzles in cosmology, provided that we assume that the standard model of cosmology is valid.
This model, although far from being perfect, is currently the simplest explanation for observations. This being said, we must keep in mind that it has defects, and may only be an effective vision of what physics truly is. It consists however of a good starting point.
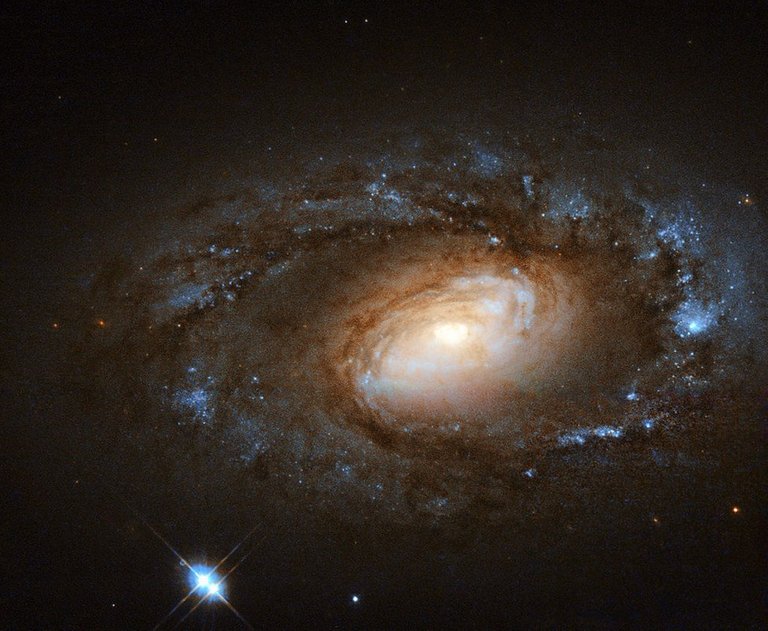
[Credits: NASA (CC BY 2.0)]
With all assumptions made so far, we can calculate the amount of dark matter in the universe for a given model, and verify that it matches observations. Moreover, we can investigate to what extent signs of dark matter can be found in cosmic rays, accelerators or in direct detection experiments.
In the first case, we analyse the amount of cosmic rays reaching Earth, and we investigate if they feature properties compatible with that emerging from the annihilation of dark matter particles elsewhere in the universe. In the second case, we accelerate high-intensity beams of particles (like at the LHC at CERN) and smash them. The associated large amount of energy allows us to produce new particles (provided they exist), and in particular dark matter. Finally, in a direct detection experiment, we try to observe dark matter particles going through our planet and hitting atomic nuclei localised in huge detectors.
For now, no sign of dark matter has however been observed, so that all means to find dark matter only led to constraints on existing models.
In the rest of this post, we make a third assumption: dark matter has a composite origin. In this case, it connects with the Standard Model through interactions with a mediator particle and the top quark, as discussed earlier in this blog.
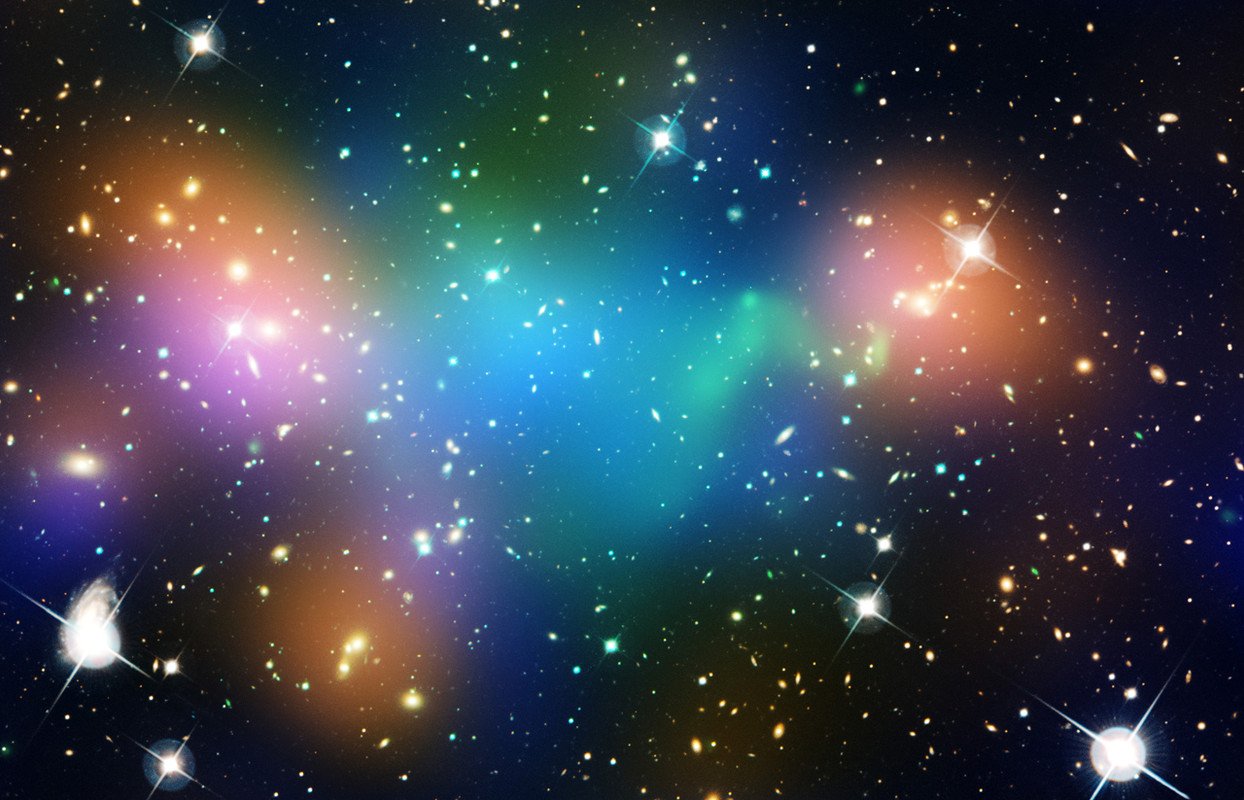
[Credits: NASA/ESA (CC BY 2.0)]
A realistic description of composite dark matter
In a previous blog, I have discussed how my collaborators and I investigated how composite dark matter was a viable option, in light of existing constraints from cosmic rays, direct detection and the LHC. It turned out that viable scenarios predicting the right amount of dark matter required a strong coupling of the dark matter to the Standard Model, and that particle colliders were the only way to have a chance to discover dark matter in the next decades.
In the follow-up article (to which this blog is dedicated), we studied the composite dark matter paradigm further, and we demonstrated that the above conclusions were too strong.
Composite dark matter models can be simply constructed by taking the Standard Model, and adding a dark matter and a mediator particle to it. Then, we need to allow the dark matter to interact with the mediator and the top quark. This is however too simplistic. Generally, composite scenarios predict that the theory should include not only one, but possibly more than one mediator, with orthogonal properties.
This is the path that we have followed in our last article: we took the previous model and added a second mediator with orthogonal properties (I won’t enter into detail here; however feel free to ask questions about this if you are interested).
Does it change a lot? Well, yes! The most dramatic impact is that the rate at which a dark matter particle could hit an atomic nucleus localised on Earth gets enhanced, by virtue of the new particle and its interactions. This means that dark matter direct detection experiments now have a chance to observe composite dark matter. Let’s illustrate this by a figure.
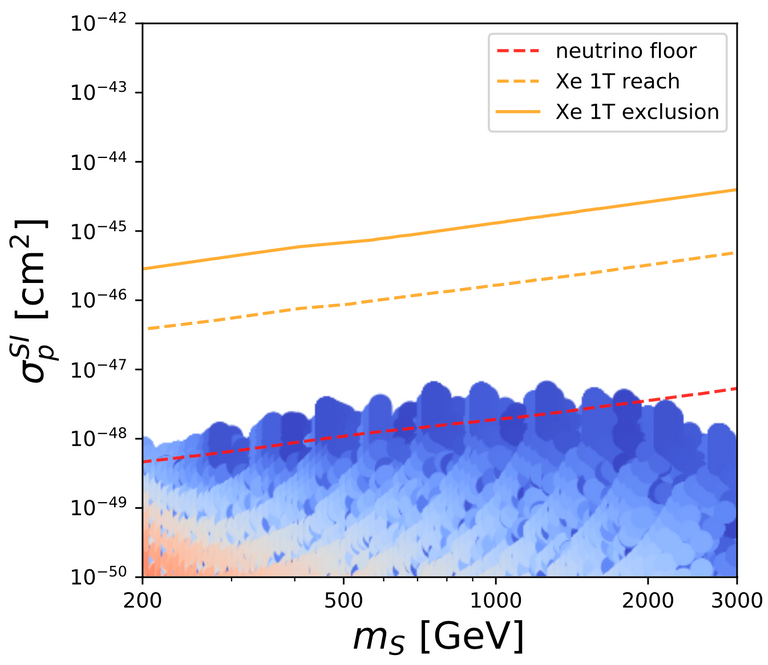
[Credits: arXiv:2209.13093 [hep-ph]]
In this figure, we report the rate at which dark matter can interact with a proton (one of the particles composing atomic nuclei). The rate is shown on the Y-axis, and we display the dark matter mass on the X-axis (the units are in GeV, with 1 GeV being the proton mass). All coloured points correspond to a viable scenario, predicting the right amount of dark matter.
The solid orange line is the current limit from dark matter direct detection experiments. Any scenario above this line is excluded as it would correspond to a signal impossible to miss (and no signal has been found). The dashed orange line corresponds to the expectation of the future upgrade of the Xenon 1T experiment. Anything above the dashed orange line could be observed or excluded very soon.
What is important is the red dashed line. It represents the so-called neutrino floor. Below this line, dark matter events are too rare to be observed from the flux of neutrinos hitting the detector. We observe that many composite scenarios lie below this line, so that future dark matter direct detection experiments have no chance to observe them.
However, we also observe that some scenarios lie above the red line. This means that we actually have options to observe a signal in future experiments. And that’s pretty good news, as this means that we have a new handle on composite dark matter models!
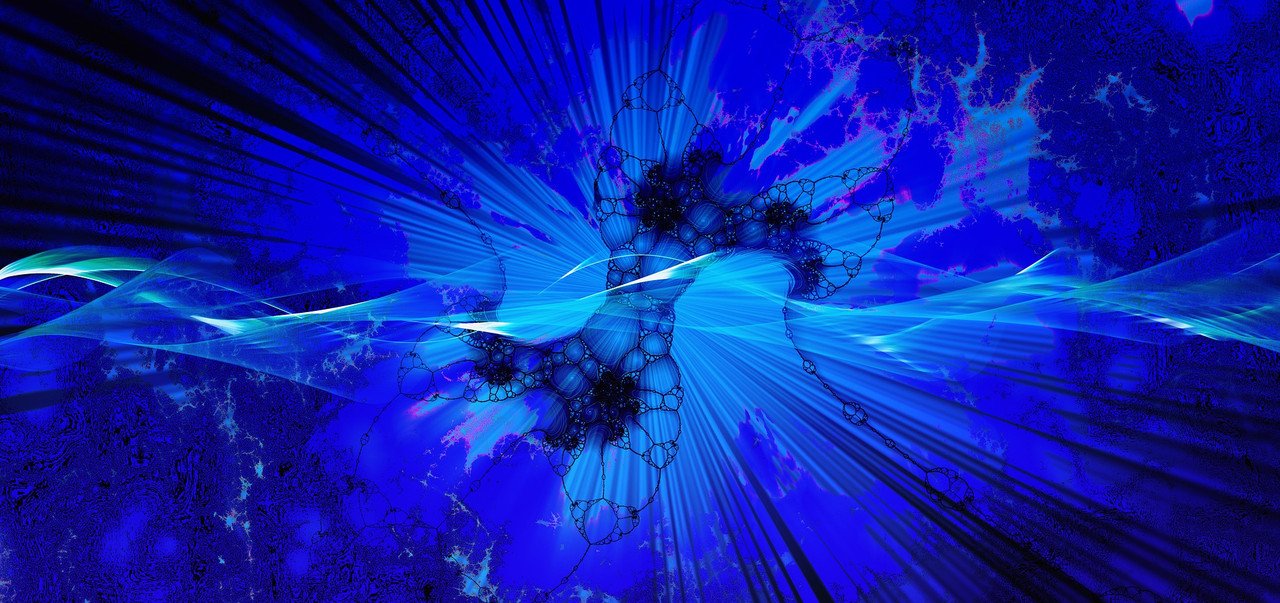
[Credits: geralt (Pixabay)]
Summary: a composite dark side brighter than expected
Composite models are a class of theories beyond the Standard Model of particle physics that assume that there are new fundamental particles (still to be observed) that are sensitive to a new strong interaction. This allows them to form a variety of new composite particles that could be observed in present and future experiments.
Among those new particles, models often predict a particle (that I called a mediator) connected to the top quark of the Standard Model. Such a particle is needed to explain the large mass of the latter. In addition, the zoo of composite particles can also include a particle that could play the role of dark matter, and that could leave signatures in particle accelerators like the LHC, cosmic rays or dark matter direct detection experiments.
In my previous work, we have explored the viability of the above scenarios, and have found that dark matter must be strongly coupled to the mediator and the Standard Model. Moreover, we have shown that the only way to observe its signs would be at particle colliders.
A few weeks ago, with my collaborators we explored further those setups, but in a situation closer to reality. In general, composite models feature several mediators relating dark matter to the Standard Model with different properties.
This more realistic description allowed us to show that next-generation dark matter direct detection experiments can be sensitive to such scenarios, and thus have some potential to observe dark matter in the future. Therefore, composite dark matter may not be as hidden as initially thought.
I stop here for today, and I hope you enjoyed reading this blog as much as I enjoyed writing it. Feel free to ask questions, comment on the text and give feedback in the comment section below. Have a nice end of the week!